By Ankit SinghReviewed by Susha Cheriyedath, M.Sc.Apr 9 2024
In modern physics, the precise manipulation and control of the temperature of atoms and molecules have unlocked numerous possibilities in fields such as quantum simulation and quantum information processing. At the intersection of quantum mechanics and optics, laser cooling emerges as a groundbreaking technique. It allows for the cooling of matter to extremely low temperatures, paving the way for advancements in these areas.1,2
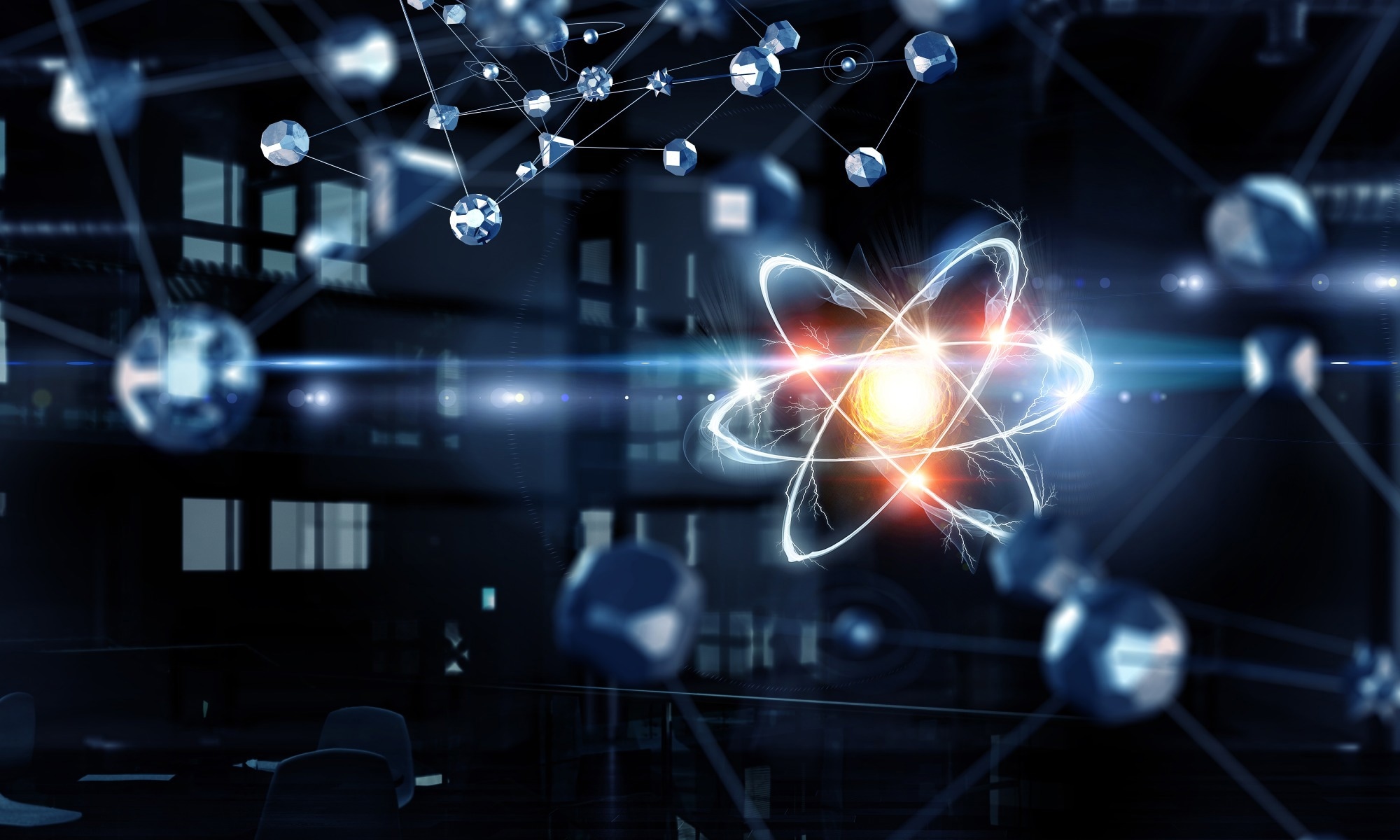
Image Credit: Sergey Nivens/Shutterstock.com
Laser cooling, the technique of lowering the temperatures of atoms, ions, or solids using laser light, was conceptualized before the laser itself was invented. It was not until the 1970s, however, that Chu S. and colleagues experimentally demonstrated this concept.
Their groundbreaking work revealed that atoms could be cooled to temperatures nearing absolute zero by precisely controlling their momentum with laser light. For their pioneering contributions to the development of laser cooling techniques, Steven Chu, Claude Cohen-Tannoudji, and William D. Phillips were awarded the Nobel Prize in Physics in 1997.2,3
This article will delve into the specifics of laser cooling and examine its principles, methodologies, and applications in quantum simulations and precision metrology, highlighting its role in advancing modern physics and technology.
Laser Cooling Methods
Laser cooling methods vary depending on the system being cooled. In gaseous systems, an atom's momentum is captured within its translational degrees of freedom, whereas in solids, this momentum is stored in the lattice vibrations, also known as phonons. Despite these differences, the overarching objective of laser cooling—to reduce the momentum of atoms using laser light—remains consistent across different systems.4
At the heart of laser cooling is the principle of Doppler cooling. This principle outlines how atoms moving toward a laser beam are able to absorb photons and then re-emit them in random directions. This process effectively reduces the system’s momentum, resulting in cooling. Beyond Doppler cooling, there are additional methods, such as Sisyphus cooling and evaporative cooling, that, when employed, enable the achievement of ultra-low temperatures.
Doppler Cooling
Doppler cooling, the foundation of laser cooling technology, relies on the interaction of atoms with laser light. This method assumes simplistic two-level systems and can be applied to neutral atoms and ions.3,4
This technique involves an atom, moving at a certain speed, encountering a laser beam tuned slightly below its resonance frequency. The atom absorbs photons due to the Doppler effect, which elevates its perceived frequency above the laser's, leading to photon absorption. Subsequent anti-Stokes fluorescence diminishes the atom's momentum, facilitating cooling. The minimum temperature achievable with this method, known as the Doppler limit, is about 100 μK.1,3
Sisyphus Cooling
Sisyphus cooling, or sub-Doppler laser cooling, builds on Doppler cooling by leveraging the hyperfine structures of atoms. It utilizes the periodic motion of atoms within an optical lattice, formed by intersecting laser beams with orthogonal polarizations. As atoms traverse this lattice, they encounter a spatially varying potential energy landscape, diminishing their momentum and consequently reducing the temperature.9 The lowest temperature achievable with Sisyphus cooling, often referred to as the recoil limit, spans from 0.1 to 1 μK.4
Evaporative Cooling
Evaporative cooling takes a distinct approach to achieve extremely low temperatures. It involves the selective removal of the hottest atoms from a trapped ultracold gas using radiofrequency or microwave techniques. This process reduces the average kinetic energy of the remaining atoms, thereby lowering the overall temperature. Evaporative cooling has been pivotal in attaining quantum degeneracy, a state where atoms exhibit collective quantum mechanical behavior.9
Applications of Laser Cooling
Atomic Clocks and Precision Measurements
Atomic clocks are essential for precise timekeeping and synchronization in a wide range of applications, such as global navigation systems and fundamental research in physics. Laser-cooled atoms are vital components in atomic clocks as they provide stability and accuracy to the atomic clocks. In recent times, laser-cooled atomic clocks have enabled crucial developments in fields like geodesy, telecommunications, and space exploration.8
Quantum Information Processing
Laser-cooled atoms and ions play an important role in the development of quantum computers and simulators. The long coherence times of laser-cooled atoms provide exquisite control for implementing quantum gates and storing quantum information. With the help of laser cooling and quantum mechanics, researchers aim to tackle complex computational problems that are beyond the capabilities of classical computers, ranging from cryptography to material science.8
Precision Metrology and Fundamental Physics
Laser cooling techniques have played a key role in understanding the laws of physics. It has enabled precise measurements of fundamental constants and properties of matter. Furthermore, with the help of laser-cooled atoms, experiments have been designed to test the principles of quantum mechanics and study the limits of gravitational and inertial forces.8 It has also played an instrumental role in exploring novel phenomena such as Bose-Einstein condensation and quantum degeneracy.12
Latest Developments and Research
Recent developments in the field of laser cooling techniques have opened up new paths for scientific investigation. One major breakthrough is the use of hybrid cooling techniques. This innovative approach not only enhances the scope of cooling across a diverse array of atomic and molecular species but also amplifies research capabilities in areas such as quantum simulation, precision spectroscopy, and quantum information processing.6,9
Recent publications have further extended the application of laser cooling techniques to complex quantum systems, including polar molecules and symmetric top molecules, broadening the horizon of potential studies.9-11
A pivotal study featured in Nature illustrates the successful trapping and sub-Doppler cooling of a polyatomic molecule, specifically calcium hydroxide (CaOH), to a temperature of 110 μK utilizing magneto-optical trapping. The results of this study position CaOH as a notably promising entity for applications within the quantum sciences domain, including but not limited to quantum simulations and computational advancements.13
Subsequent research, also published in Nature, has led to the development of an optical-tweezer array for the manipulation of ultracold polyatomic molecules. his array not only facilitates precise control over the molecules' internal quantum states but also introduces a non-destructive imaging capability with enhanced efficiency.14 This advancement is poised to revolutionize the approach to molecular manipulation and observation.
The convergence of interdisciplinary research efforts has catalyzed the development of integrated platforms that combine laser cooling with atom-trapping technologies. These innovations have culminated in the creation of compact, portable cold atom devices. Such devices are set to redefine field applications across a spectrum of uses, including inertial sensing, quantum communication, and navigational systems.3,5
Challenges
Despite the advances in laser cooling technology, significant challenges remain in the way of achieving its full potential. Complex experimental setups and the need for precise control over experimental parameters pose major challenges in the implementation of laser cooling technology.1
Additionally, external parameters like stray light, interaction with residual gases, and magnetic fields lead to the decoherence of trapped atoms, which decreases the technique's efficiency.2-4 Scaling up laser cooling techniques to larger systems or complex molecules remains a formidable challenge, requiring innovative approaches to overcome technical limitations and scalability issues.10,11
Future Perspectives and Conclusion
Recent advancements in laser technology, quantum optics, and materials science have set the stage for an exciting future in laser cooling technology. The key to unlocking this potential lies in enhancing the efficiency, scalability, and versatility of laser cooling methods. Such improvements promise not just groundbreaking discoveries but also innovations that could redefine technological frontiers.
Integrating laser-cooled atoms and ions with burgeoning quantum technologies, such as quantum networks and sensors, holds the promise of transforming communication, sensing, and computation. Moreover, pioneering novel cooling mechanisms and exploring complex quantum states could significantly deepen our understanding of the quantum world.9,10
The current progress in laser cooling technology is paving the way for revolutionary applications in ultra-cold matter-based technologies. These advancements are not just expanding our capabilities in precision measurement but also offering unparalleled opportunities to explore the mysteries of quantum mechanics.10
In summary, laser cooling is transcending the boundaries of theoretical physics, venturing into quantum mechanics, precision metrology, and quantum computing. It signifies a future where leveraging the coldest temperatures could lead to the most significant discoveries, pushing the limits of what is scientifically and technologically achievable.
References and Further Reading
- Seletskiy, D. et al. (2016). Laser cooling in solids: advances and prospects. Reports on Progress in Physics,79, 096401
- Phillips, W. D. (1998). Nobel Lecture: Laser cooling and trapping of neutral atoms. Reviews of Modern Physics, 70(3), 721–741. https://doi.org/10.1103/RevModPhys.70.721
- McCarron, D. (2018). Laser cooling and trapping molecules. Journal of Physics B: Atomic, Molecular and Optical Physics, 51(21), 212001. https://doi.org/10.1088/1361-6455/aadfba
- Nemova, G. (2019). Field Guide to Laser Cooling Methods. SPIE Field Guides FG45
- Karl, R., Yin, Y., & Willitsch, S. (2023). Laser cooling of trapped ions in strongly inhomogeneous magnetic fields. Molecular Physics. https://doi.org/10.1080/00268976.2023.2199099
- Zhang, C., Rittenhouse, S. T., Tscherbul, T. V., Sadeghpour, H. R., & Hutzler, N. R. (2024). Sympathetic Cooling and Slowing of Molecules with Rydberg Atoms. Physical Review Letters, 132(3). https://doi.org/10.1103/physrevlett.132.033001
- Thompson, J. K., et al. (2017). Sympathetic Cooling of Atomic Ions with Multispecies Doppler Cooling of Neutral Atoms. Physical Review Letters, 119(21), 213002. https://doi.org/10.1103/PhysRevLett.119.213002
- Ye, J., & Katori, H. (2017). Optical Lattice Clocks and Quantum Metrology. arXiv preprint, 1708.00410. https://arxiv.org/abs/1708.00410
- Zhang, X., et al. (2020). Cooling ultracold polar molecules in deep optical lattices. Science, 369(6506), 1355–1359. https://doi.org/10.1126/science.abb4218
- Mitra, D., Vilas, N. B., Hallas, C., Anderegg, L., Augenbraun, B. L., Baum, L., Miller, C., Raval, S., & Doyle, J. M. (2020). Direct laser cooling of a symmetric top molecule. Science, 369(6509), 1366–1369. https://doi.org/10.1126/science.abc5357
- Augenbraun, B. (2021) Methods for Direct Laser Cooling of Polyatomic Molecules. Harvard University Cambridge, Massachusetts.
- Schreck, F., et al. (2001). Quasipure Bose-Einstein Condensate Immersed in a Fermi Sea. Physical Review Letters, 87(8), 080403. https://doi.org/10.1103/PhysRevLett.87.080403
- Vilas, N. B., Hallas, C., Anderegg, L., Robichaud, P., Winnicki, A., Mitra, D., & Doyle, J. M. (2022). Magneto-optical trapping and sub-Doppler cooling of a polyatomic molecule. Nature, 606(7912), 70–74. https://doi.org/10.1038/s41586-022-04620-5
- Vilas, N. B., Robichaud, P., Hallas, C., Li, G. K., Anderegg, L., & Doyle, J. M. (2024). An optical tweezer array of ultracold polyatomic molecules. Nature. https://doi.org/10.1038/s41586-024-07199-1
Disclaimer: The views expressed here are those of the author expressed in their private capacity and do not necessarily represent the views of AZoM.com Limited T/A AZoNetwork the owner and operator of this website. This disclaimer forms part of the Terms and conditions of use of this website.