Article updated by Rebecca Ingle on 11 January 2022
The laser (Light Amplification by Stimulated Emission of Radiation) is one of the most ubiquitous and versatile pieces of technology in the modern world. They are a crucial technology in fields as diverse as data storage, vision-correcting surgery, displays, metrology, and even nuclear fusion and quantum computing research.
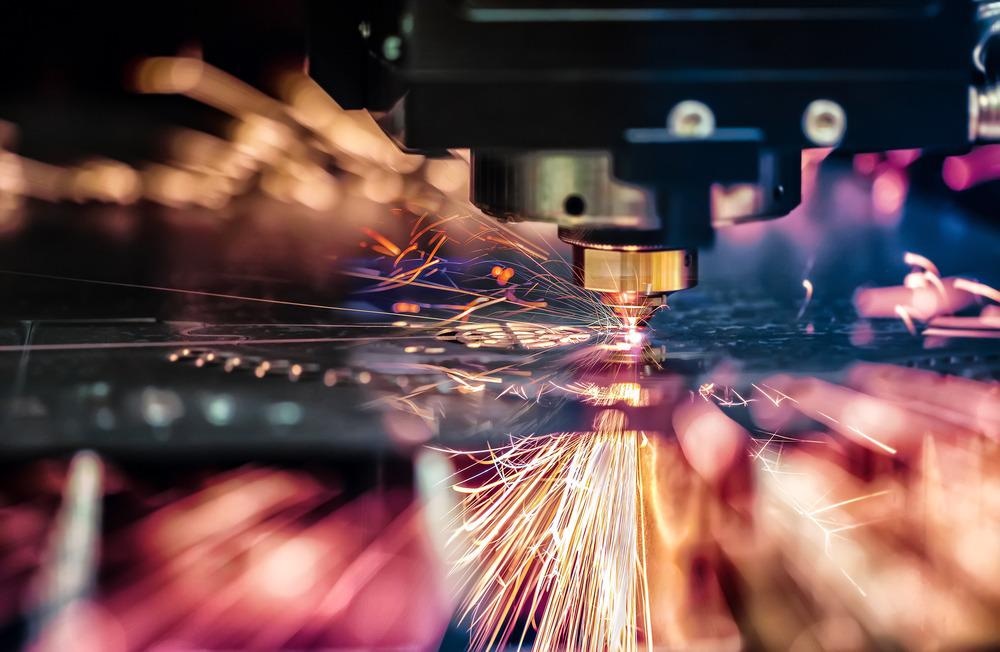
Image Credit: Andrey Armyagov/Shutterstock.com
Lasers use a process called "stimulated emission" in a gain medium to produce a beam of coherent light, which means that the light waves which make up the beam oscillate perfectly in sync.
One of the key advantages of the spatial coherence of laser beams is that they remain collimated over long distances and can be focused to very small spot sizes, giving high local powers that can be used for applications such as laser cutting. The special properties of laser light open up many applications that ordinary light is unsuitable for.
How lasers work (in theory)
Figure 1. Minute Physics outline the basic principles of lasers, and explain how stimulated emission works.
Discovery of Lasers
The concept of using stimulated emission to generate a beam of tightly focused, coherent electromagnetic radiation was first brought to realization in 1954, although our understanding of the physical principles involved had been developing for some decades before that point, beginning with Einstein in 1917.
Charles Townes at Columbia University in New York, and, independently, Dr. Basov and Dr. Prochorov at the Lebedev Institute in Moscow, developed the first "MASER" - effectively a laser which emits microwave radiation instead of visible light. All three scientists were awarded with a Nobel Prize.
By 1960, the available technology had progressed far enough to allow the first visible-light laser to be developed - the energy required to excite a light-emitting material is much higher than the amount required for a maser. Theodore Maiman built the first ruby laser at the Hughes Research Laboratories, which emitted a pulsed beam of laser light at 694.3 nm.
Structure and Operation of a Laser
The most basic laser consists of a gain medium within an optically resonant cavity with a mirror at both ends.
When the particles in the gain medium are energized by an electrical current, an ordinary light source, or even another laser, the particles in the gain medium are pumped into a temporary excited state. When this state decays, the particle emits a photon of a specific wavelength.
With a sufficiently efficient pumping scheme to produce an excited state in the gain medium, a very large number of photons of the same wavelength can be produced. These photons can then interact with an excited state to stimulate the emission of a second photon, normally at another wavelength. All the photons produced in this stimulated emission will be coherent.
The frequency of the emitted laser light is dependent on the electronic structure of the gain medium, which can be a crystal, a semiconductor, a gas, or even a coloured liquid. The material used for a particular laser depends on the required light properties for the application.
The gain medium is normally placed in an optical cavity; a system of mirrors reflects the emitted light backwards and forward through the medium, increasing its intensity with each pass.
One of the mirrors at the end of the laser cavity is semi-silvered - this reflects most of the light back into the cavity (typically 1 - 5%), while letting some of it exit. Most laser designs include some type of controllable optical switch, which only allows the light to leave the cavity once the gain has become saturated.
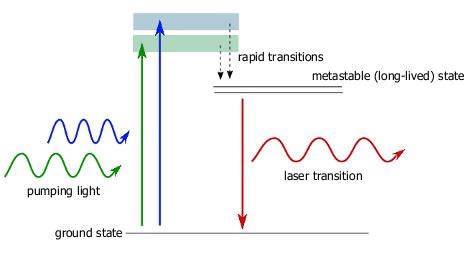
Figure 3. Energy level diagram for a ruby laser. Blue and green light from the broad-spectrum pumping light excites electrons in the ruby's chromium ions to broad 4F states. The electrons then decay via a metastable 2E doublet state to produce photons with a wavelength of 694.3 nm.
Table 1. Some common laser materials and their output wavelengths
CO2 |
Gas |
10.6 µm |
Surgery, laser cutting/marking, welding |
InGaAsP / InP |
Semiconductor |
1000-1650 nm |
Fibre optic communications |
Titanium-doped sapphire |
Crystal |
650-1100 nm |
Spectroscopy, photonics, research |
Ruby |
Crystal |
694.3 nm |
Rangefinding, holographic materials testing |
AlGaInP/GaAs |
Semiconductor |
635-670 nm |
DVD players, laser pointers |
He-Ne |
Gas |
632.8 nm |
Alignment, interferometers |
Rhodamine 6G |
Dye |
566 nm |
Tuneable lasers, spectroscopy |
InGaN/GaN on SiC |
Semiconductor |
380- 470 nm |
Data storage |
Pulsed vs. Continuous Wave Lasers
Pulsed Lasers
In a pulsed laser system, such as a ruby laser or Ti:sapphire laser, short pulses of light are emitted from the optical cavity. There are a variety of methods to pulse a laser system and the choice depends on the temporal duration and properties of the pulses required. For example, ultrashort laser pulses are typically generated using mode-locking schemes and are femtoseconds or picoseconds in duration.
Using shorter pulse durations also leads to higher peak powers which can be advantageous for power-demanding applications such as multiphoton microscopy and strong-field physics experiments. For fusion reactions, where over trillions of Watts of power are needed, pulsed laser schemes can help achieve these peak powers.
Continuous Wave Lasers
Some lasers can operate in a continuous mode, emitting a constant light intensity rather than a pulse train. The most common continuous wave lasers are helium-neon lasers and some dye lasers. Not all gain media are amenable to continuous operation, however. The laser must be constantly recharged, which could damage the material if the power required to keep the laser charged is too high.
Future Developments of Laser Technology
New types of laser systems are in constant development. Recent advances in fiber-based laser systems have made it possible to achieve very high repetition rates without sacrificing pulse durations and powers. Fiber lasers can also produce very high powers necessary for laser machining and engraving.
With photonics technologies becoming more widespread, there is a greater need for highly stable lasers systems, particularly for use in optical clocks, quantum computers, and similar technologies.
The telecommunications industry has a particular interest in the development of robust mid-infrared laser systems. As lasers find an ever-growing list of applications, there seems to be no sign of a slowdown in the development of new laser technologies to achieve this.
References and Further Reading