Optical waveguides are specialized devices or mediums designed to confine and direct light propagation along a predetermined path, primarily in the form of optical signals or photons. These waveguides rely on the fundamental principles of electromagnetic theory to regulate the transmission and containment of light waves within their structures.
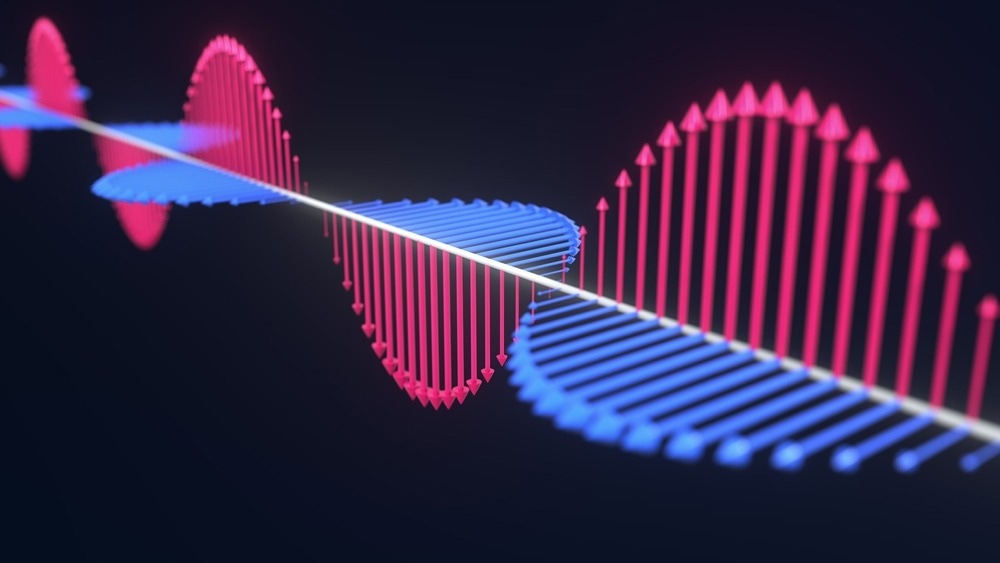
Image Credit: Irina Anosova/Shutterstock.com
Optical waveguides are comprised of a core that confines light and a surrounding cladding or substrate. The core's refractive index is greater than that of the cladding, which means that light rays entering the waveguide core undergo total internal reflection at the interface, confining them inside the core. This enables guided transmission of signals over extended distances.
The significance of researching optical waveguides lies in their profound impact on our daily lives, primarily driven by the remarkable progress in fiber optics technology and optical communications. Beyond communications, optical waveguides are integral in various sensing, imaging, and medical technologies. For example, optical waveguides have become indispensable in advancing biomedical imaging devices and fiber-based endoscopes in biophysics and life sciences.
With their versatility and growing utility across scientific domains, optical waveguides promise to transform how we transmit and leverage light further across industrial, medical, and scientific frontiers.
Governing Electromagnetic Theory and Equations in Optical Waveguides
Understanding the operation of optical waveguides hinges on the fundamental concepts and equations derived from electromagnetic wave theory. Based on Maxwell's equations, these principles are pivotal in deciphering how electromagnetic waves behave within optical waveguides.
The following two Maxwell's curl equations are key to analyzing wave propagation within optical waveguides, outlining how electric and magnetic fields evolve and interact in both space and time.
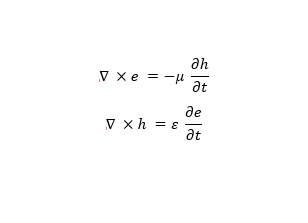
Here, e = electric field, h= magnetic field, ε = the permittivity and μ is the permeability of the optical waveguides.
By combining Maxwell's equations with the boundary conditions, the modes or field patterns that can propagate in the optical waveguides can be determined through eigenvalue equations. Each guided mode has a specific propagation constant and field distribution.
The number of modes depends on factors like waveguide dimensions, wavelength, and refractive index contrast. Single-mode optical waveguides only permit the fundamental mode, while multimode types allow multiple modes. Understanding the modal behaviors through electromagnetic simulations enables designing optical waveguides with desired properties like single-mode, polarization-maintaining, or dispersion management.
The Poynting vector (S = e × h), derived from the electromagnetic field intensities, quantifies the power flow density within the waveguide and reveals how power propagates through it. Its time average enables power flow analysis in alternating fields, contributing to a comprehensive understanding of power transmission within optical waveguides.
These core concepts and equations collectively provide the foundation for comprehending the intricate workings of optical waveguides, facilitating their practical application in various optical systems.
Emerging Trends in Optical Waveguides
The field is shifting towards novel optical waveguides with enhanced properties like low transmission losses, flexibility, and multifunctionality. For example, hollow-core photonic crystal fibers filled with air rather than glass provide ultra-low loss optical transmission. Advances in materials science allow thinner, flexible optical waveguides to be integrated with wearable technologies.
Conventional silica glass optical fibers, widely used in telecommunications, lack biocompatibility when interacting with human tissues. However, new biodegradable and flexible polymer optical waveguides efficiently transmit light and are non-reactive when introduced into the human body. These biocompatible optical waveguides are evolving various photomedical techniques such as photodynamic therapy (PDT), photothermal therapy (PTT), physiological signal detection, optical imaging, and optogenetics.
Subwavelength metamaterials are revolutionizing the creation of optical meta-waveguides, offering enhanced control over light confinement and propagation on the nanoscale. Integrating functional subwavelength structures with dielectric and plasmonic waveguide platforms unlocks many possibilities, such as versatile coupling interfaces, on-chip optical signal processing, photonic neural networks, and quantum and nonlinear devices.
While still in its early stages, optical meta-waveguide technology holds significant promise for shaping the future of photonic integrated circuits and emerging applications.
Recent Research and Development
Two-Dimensional Optical Waveguides
Researchers at the University of Chicago led by Professor Jiwoong Park developed an innovative two-dimensional optical waveguiding system using thin glass crystal sheets merely a few atoms thick. Surprisingly, this ultrathin optical waveguide delivered data over a thousand times further than similar light-based systems. The results are published in the journal Science.
Unlike current optical waveguides, where photons are enclosed within the waveguide, this system allows part of the photon to stick out of the crystal as it travels. This approach is similar to setting suitcases on a conveyor belt rather than sending them through a tube.
The open-air conveyance makes manipulating light with lenses or prisms easier and offers opportunities for microscopic sensors. "For example, say you had a sample of liquid, and you wanted to sense whether a particular molecule was present. You could design it so that this waveguide travels through the sample, and the presence of that molecule would change how the light behaves." Jiwoong Park, Lead Study Author.
This research offers the potential to advance on-chip photonic systems for communication, computing, and sensing by exploring 2D nanophotonics.
Ultra-Thin Optical Waveguides for Minimally Invasive Biomedical Imaging
In a study published in Optical Materials Express, researchers designed optical waveguides thinner than a speck of dust with minimal light loss, making them ideal for biomedical applications.
They used phenylacetylene to create the optical waveguides through laser direct writing, omitting the need for a photoinitiator, thus enhancing biocompatibility for potential implantable sensors.
"To the best of our knowledge, these are the smallest optical waveguides ever created in polydimethylsiloxane, or PDMS. Our flexible waveguides could be integrated into microfluidic lab-on-a-chip systems to eliminate bulky external optics needed to perform blood tests, for example." Ye Pu, Co-Author of the study.
The researchers are actively working to enhance the fabrication process and envision creating flexible endoscopes with these optical waveguides, enabling imaging in hard-to-reach areas within the body for diagnostic or minimally invasive surgical purposes.
Future Outlooks
Future research on optical waveguides will likely emphasize material development to enhance light transmission efficiency, explore functional materials for sensing, and optimize waveguide structures for multifunctionality, including microfluidic channels. Advancements in manufacturing and encapsulation technologies will improve waveguide performance and integration of versatile modules.
More from AZoOptics: Wavefront Analysis for High-Resolution Imaging Systems
References and Further Reading
Calvo, M. L., & Lakshminarayanan, V. (Eds.). (2018). Optical waveguides: from theory to applied technologies. CRC press. https://www.routledge.com/Optical-Waveguides-From-Theory-to-Applied-Technologies/Calvo-Lakshminarayanan/p/book/9780367389536
Lee, M., Hong, H., Yu, J., Mujid, F., Ye, A., Liang, C., & Park, J. (2023). Wafer-scale δ waveguides for integrated two-dimensional photonics. Science, 381(6658), 648-653. https://doi.org/10.1126/science.adi2322
Meng, Y., Chen, Y., Lu, L., Ding, Y., Cusano, A., Fan, J. A., ... & Ni, X. (2021). Optical meta-waveguides for integrated photonics and beyond. Light: Science & Applications, 10(1), 235. https://doi.org/10.1038/s41377-021-00655-x
Okamoto, K. (2006). Chapter 1 - Wave theory of optical waveguides - Fundamentals of optical waveguides. Elsevier. https://doi.org/10.1016/B978-012525096-2/50002-7
Panusa, G., Pu, Y., Wang, J., Moser, C., & Psaltis, D. (2019). Photoinitiator-free multi-photon fabrication of compact optical waveguides in polydimethylsiloxane. Optical Materials Express, 9(1), 128-138. https://doi.org/10.1364/OME.9.000128
Wang, J., & Dong, J. (2020). Optical waveguides and integrated optical devices for medical diagnosis, health monitoring and light therapies. Sensors, 20(14), 3981. https://doi.org/10.3390/s20143981
Disclaimer: The views expressed here are those of the author expressed in their private capacity and do not necessarily represent the views of AZoM.com Limited T/A AZoNetwork the owner and operator of this website. This disclaimer forms part of the Terms and conditions of use of this website.