Cyanobacteria have evolved complicated mechanisms to transform light energy into chemical energy during three billion years of existence. Cyanobacteria produce reducing power (NADPH) and chemical energy (ATP) for carbon dioxide fixation using only the most basic of ingredients: light and water.
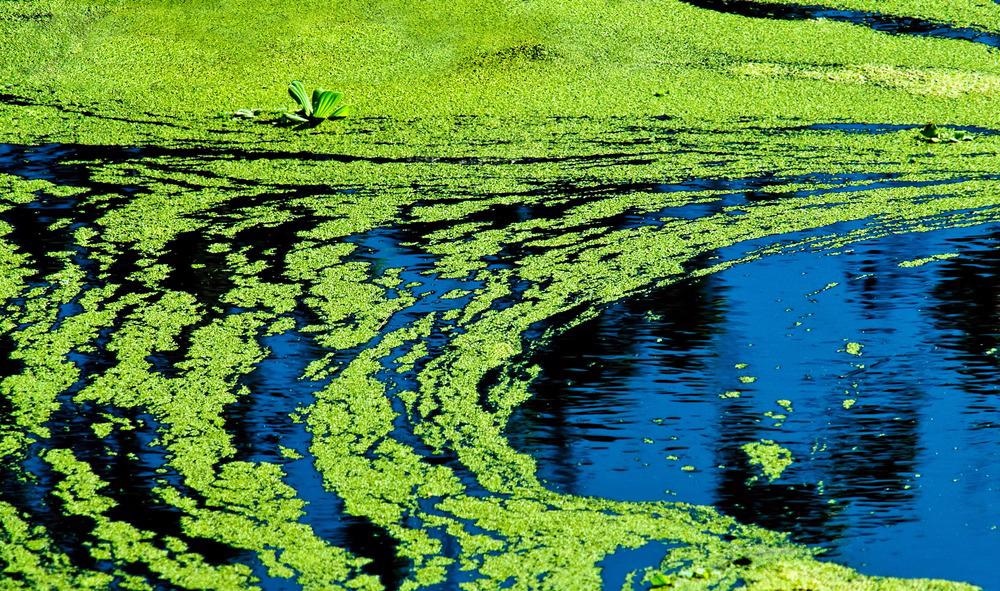
Image Credit: Alexlky/Shutterstock.com
Photosynthesis can be hampered by a process known as photoinhibition at greater light intensities (LI). Despite decades of research highlighting cyanobacteria’s potential, photoinhibition is one element that reduces their appeal as a source of low-cost organic goods and biofuels.
Photosystem II (PSII) and photosystem I (PSI) are the two primary light-activated complexes that regulate the photosynthetic process (PSI). The photosynthetic linear electron transport chain (PETC) is depicted in Figure 1 along with the electrochemical potential of the chain’s components. According to standard hydrogen potential (SHE), all electrochemical potentials presented are midpoint potentials (Eo′, V) at pH 7.

Figure 1. Schematic of the biological components of the photosynthetic electron transport chain (PETC) juxtaposed with the corresponding midpoint potentials versus standard hydrogen electrode (SHE) potentials with green arrows representing electron flow and blue arrows representing proton movement. As depicted in red, the PSII D1 subunit is subject to damage by reactive oxygen species when charge separation does not move forward fast enough in the presence of oxygen, producing singlet oxygen. PSII may be replaced with an electron delivery from duroquinol to the plastoquinone (PQ) pool. Image Credit: Lewis, et al., 2022
PSII is genetically eliminated from the cells in this study, and researchers employ a cathode-mediator device to shuttle electrons between the cell and the electrode, preventing PSII’s oxidative-photo damage, which is an extension of the research team’s earlier intellectual property.
Since this strategy needs the delivery molecule to be isoelectrically comparable to QB (−0.12 V) or at least have a potential more negative than the cyt b6f pocket (0.30 V), the yellow arrow in Figure 1 denotes a targeted position for an alternate electron source.
Methodology
Figure 2 shows the MEPS reactor, which was a normal three-electrode H-cell reactor. An Ag/AgCl reference electrode (BASi) and a cathode were on one side.

Figure 2. H-cell reactors used for MEPS where the cathode measured light-dependent current density via chronoamperometry (−0.249 V). The ΔpsbB cells in the cathodic chamber acted as a bioanode. The black device represents the laboratory lamps used for exposing reactors to light. Image Credit: Lewis, et al., 2022
The incident light intensity was changed as needed for the experiment by placing a light source directly in front of the glass reactors/cathode.
A MEPS reactor was cleaned, disinfected via autoclaving or UV exposure, and then installed in a laminar flow hood under strict sterile conditions in preparation for an investigation.
CV was first employed in the MEPS reactors to calculate the cathode potential setting that would be used in chronoamperometry tests in BG-11 with the cells present.
The ability of the mediator to transfer electrons intercellularly to the PETC was confirmed using Joliot-type spectroscopy (JTS) with Biologic JTS-10 equipment.
Results and Discussion
To figure out how to artificially stimulate electron flow in the absence of PSII, MEPS was investigated in the presence and absence of living cells that lacked PSII. These findings show that Synechocystis without PSII can behave as a bioanode by receiving electrons from an external cathode. Figure 3 shows an example of light/dark cycles with a LI of 940 μmol photons m–2 s–1.
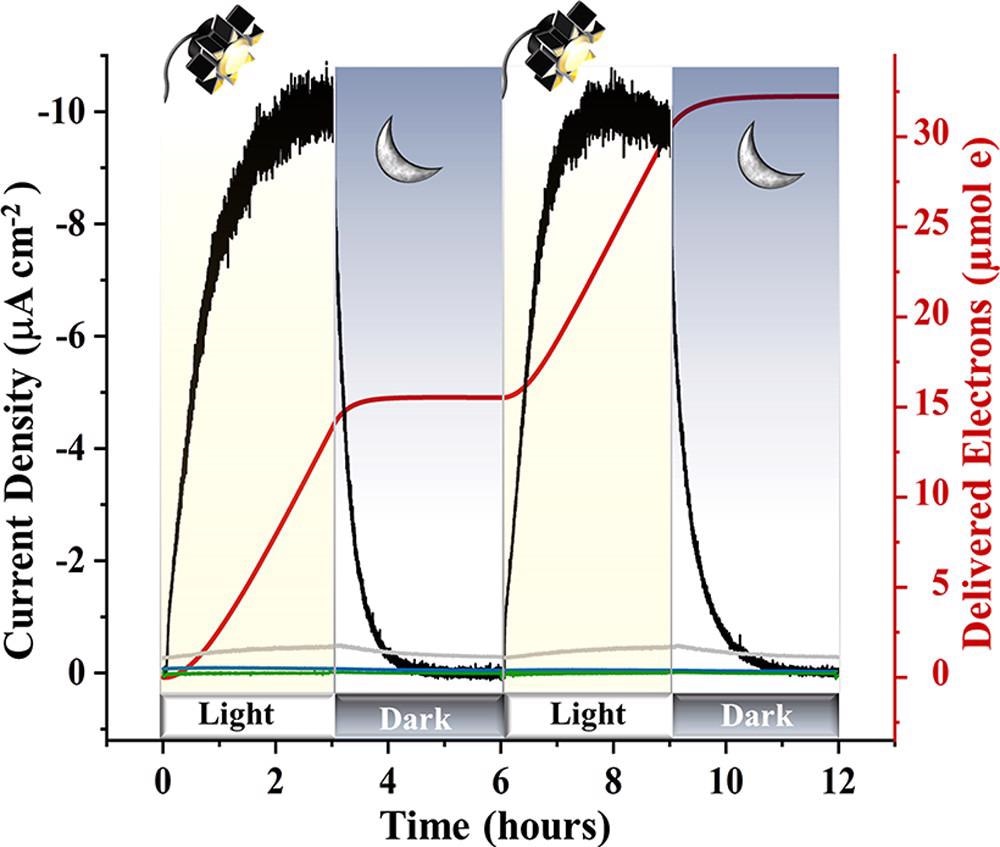
Figure 3. Electrochemical characterization of MEPS is shown. Chronoamperometry measurements (at a bias of −0.249 V vs SHE) of DQH2-mediated ΔpsbB cells were recorded for 3 h light/dark cycles, where the current density reached −10 μA cm–2 for each light cycle and delivered 15 μmol of electrons. The baseline (0.07 μA cm–2), established with DQH2 and ΔpsbB cells for a 7 h period in the dark, was subtracted out to reflect a starting value of zero. Mediatorless live ΔpsbB (green), mediatorless heat-killed ΔpsbB (blue), and heat-killed ΔpsbB cells with DQH2 added (gray) generated negligible response. Image Credit: Lewis, et al., 2022
Under a bias of −0.249 V, findings from 940 mol photons m–2 s–1 light exposure (Figure 3) show that consistent results may be achieved during the first two light/dark cycles.
To acquire a better grasp of how PSI can work in the absence of PSII, researchers tested MEPS under light circumstances when photodamage and downregulation are known to occur. Figure 4 illustrates this.
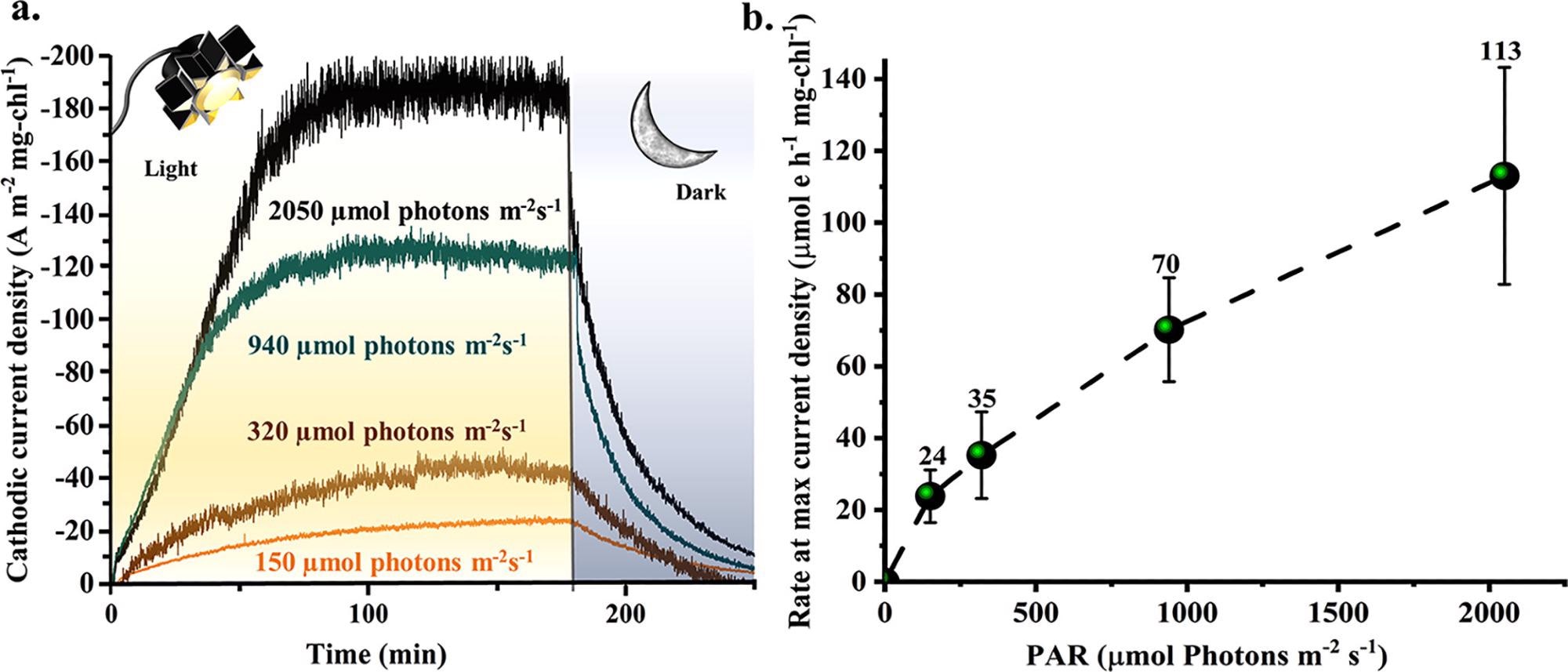
Figure 4. (a) Individual light/dark cycle examples for four Lis: 150 (orange), 320 (brown), 940 (teal), and 2050 (black) μmol photons m–2 s–1 normalized to mg-chl (at −0.249 V vs SHE). (b) Triplicate studies determining the MEPS light-activated electron delivery rate in 50 mL reactors at maximum current density levels (which reached those points at different times). The following Lis were tested: 150, 320, 940, and 2050 μmol photons m–2 s–1 and resulted in electron delivery rates of 24, 35, 70, and 113 μmol e h–1 mg-chl–1, respectively. Image Credit: Lewis, et al., 2022
As illustrated in Figure 4b, triplicate investigations compared the maximal electron delivery rate generated during the first three light cycles.
For the first three light cycles, Figure 5 compares current densities after a 1.5-hour exposure to light (within the first 24 hours). Figure 6 depicts the rates of JTS-based re-reduction.
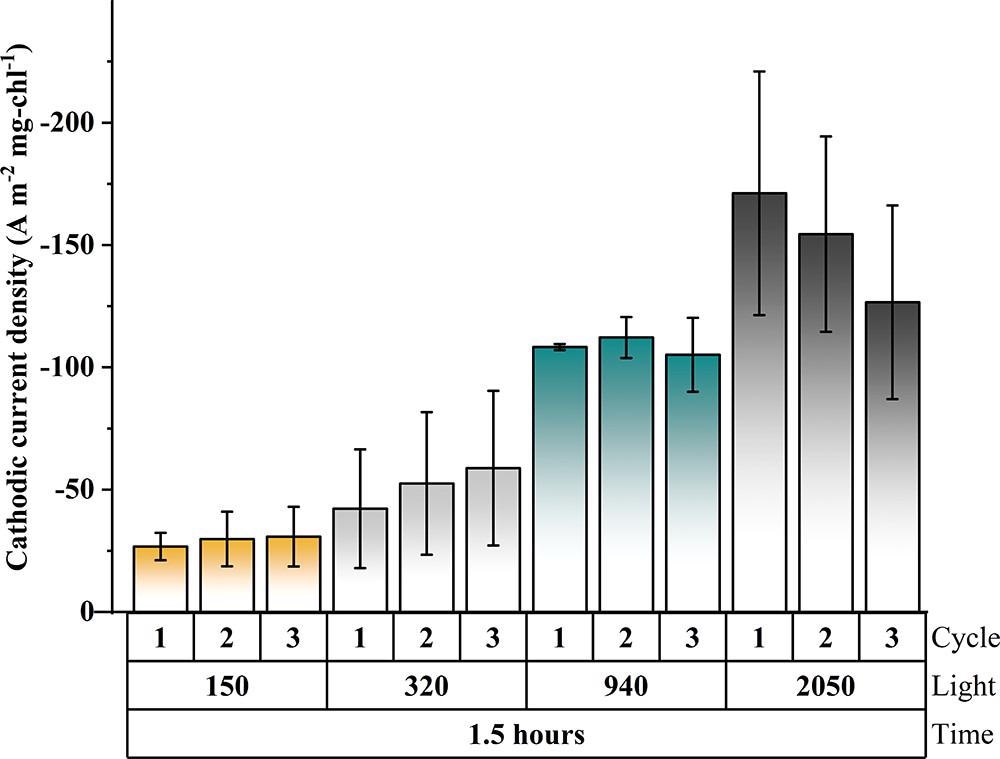
Figure 5. Bar diagram depicting triplicate results of current density generated in the first 1.5 h of three consecutive light cycles for four light intensities (at −0.249 V vs SHE): 150 (orange), 320 (gray), 940 (teal), and 2050 (black) μmol photons m–2 s–1. Image Credit: Lewis, et al., 2022
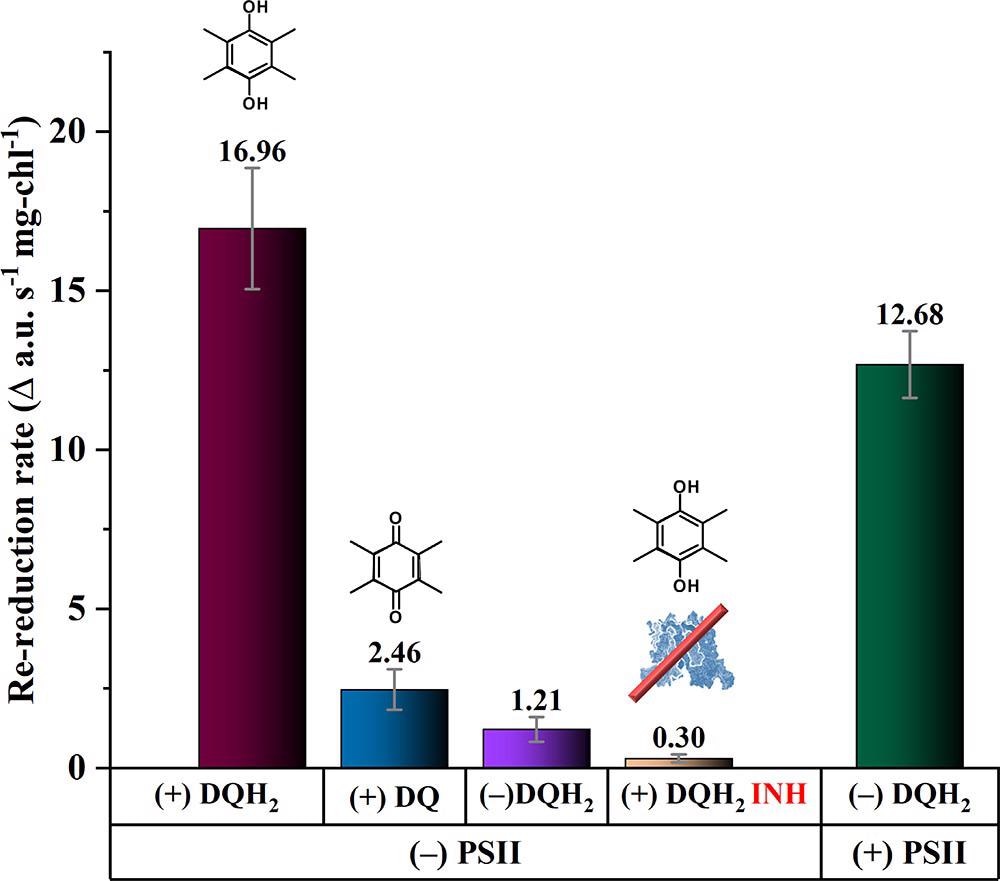
Figure 6. Joliot-type spectroscopy absorbance kinetics showing the re-reduction rate (absorbance units per s normalized to chlorophyll = a.u. s–1 mg-chl–1) of P700+ → P700 when exposed to 940 μmol photons m–2 s–1 of actinic light. Samples tested: ΔpsbB cells with reduced mediator (burgundy), ΔpsbB cells with oxidized mediator (blue), ΔpsbB cells lacking mediator (violet), PSII-less cells with reduced mediator with DBMIB, a cyt b6f inhibitor (orange) and wild-type cells (green). Image Credit: Lewis, et al., 2022
Conclusion
MEPS reactors were created with the goal of allowing photosynthetic electron transport to occur without being hampered by PSII photodamage. Figure 7 depicts the interpretation of the system’s operation.
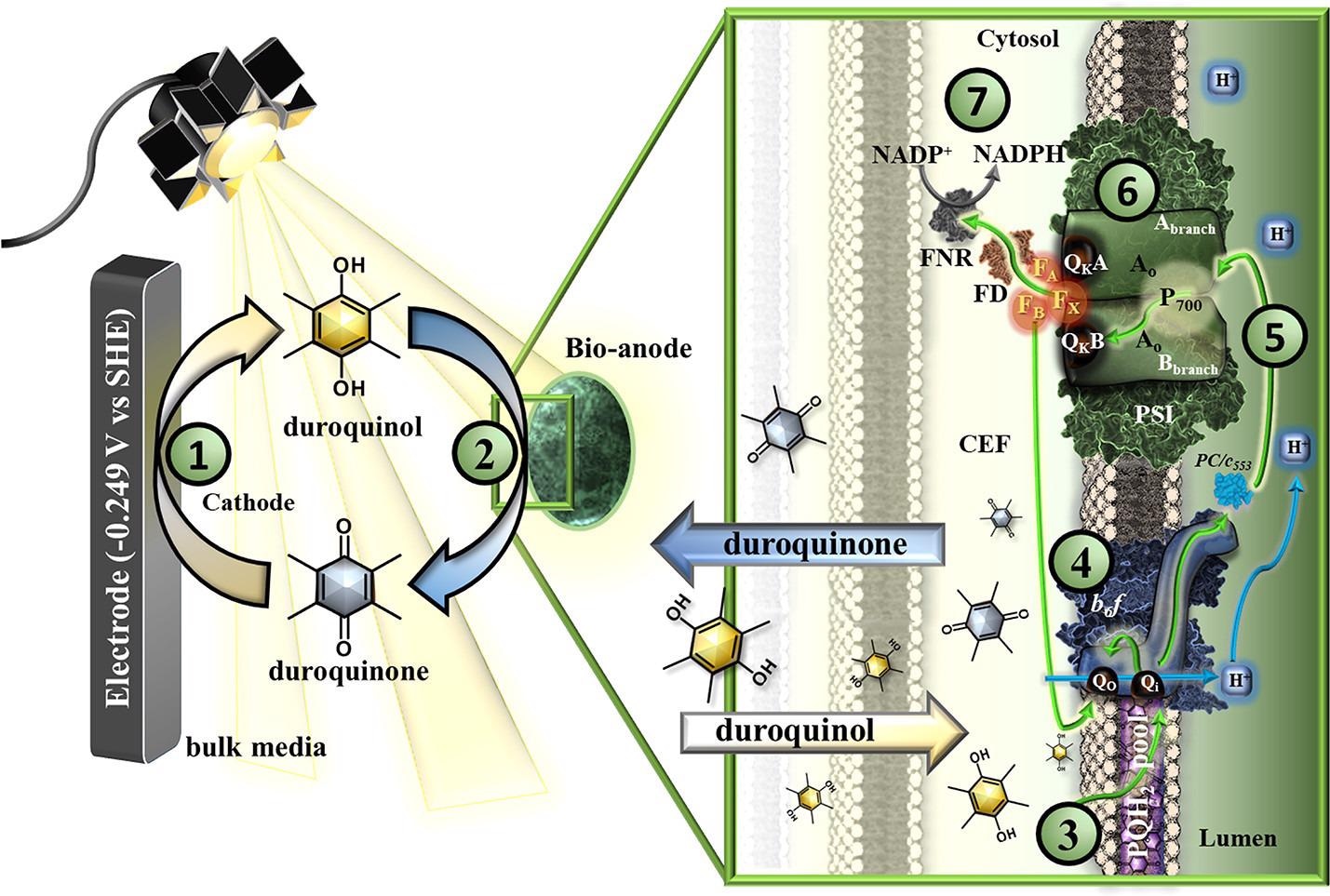
Figure 7. Schematic of the microbial electro-photosynthetic system (MEPS) with green arrows representing electron flow and blue arrows representing proton movement. (1) Molecular mediator (duroquinone to duroquinol) reduction at the cathode −0.249 V vs SHE). (2) Live ΔpsbB cells act as bioanodes where the oxidation of the mediator occurs. (3) Within the cells, at the interface of the thylakoid membrane, duroquinol mediators donate electrons to (4) the cyt b6f complex, releasing protons into the luminal space and passing electrons further down the chain; (5) cytochrome c553 or plastocyanin then transport the electrons to Photosystem I. (6) Upon exposure to light, PSI catalyzes the light-driven electron transfer from P700 to the cytoplasmic site of the membrane where it reduces (7) ferredoxin (FD) that shuttles electrons to FNR for the reduction of NADP+ to NADPH. A proton gradient is generated in the process, which drives ATP synthesis. If NADP+ is not available electrons will be shuttled back into the PETC via cyclic electron flow (CEF) to the cyt b6f complex or may be dissipated via Flv1/Flv3 that reduces enzymes or redox regulation pathways in the cell (for clarity not depicted in the figure). Image Credit: Lewis, et al., 2022
The MEPS cathode started photosynthesis in the first stage by electrochemically controlling the reduction of quinone-type redox mediators in the bioreactor’s bulk medium. Exogenous mediators were able to effectively feed electrons to the thylakoid interface at rates comparable to or higher than PSII in wild-type cells and were provided to the PETC prior to cyt b6f, enabling proton pumping and ATP synthesis, according to JTS data.
These findings suggest that MEPS could be utilized to improve PETC at high light intensities (for example, for biotechnological applications), as well as to investigate additional photoinhibitory processes in the lack of PSII, as well as light-driven electron flow via PSI without PSII confounding effects.
Journal Reference
Lewis, C. M., Flory, J. D., Moore, T. A., Moore, A. L., Rittmann, B. E., Vermaas, W. F. J., Torres, C. I., Fromme, P. (2022) Electrochemically Driven Photosynthetic Electron Transport in Cyanobacteria Lacking Photosystem II. Journal of the American Chemical Society, 144(7), pp. 2933–2942. Available Online: https://pubs.acs.org/doi/10.1021/jacs.1c09291.
References and Further Reading
- Blankenship, R E (2014) Molecular Mechanisms of Photosynthesis, 2nd ed.; Wiley.
- Singh, J. S., et al. (2019) Cyanobacteria: A sustainable and commercial bio-resource in production of bio-fertilizer and bio-fuel from waste waters. Environmental and Sustainability Indicators, 3–4(1), pp. 1–8. doi.org/10.1016/j.indic.2019.100008.
- Lau, N. S., et al. (2015) Cyanobacteria: Photoautotrophic Microbial Factories for the Sustainable Synthesis of Industrial Products. BioMed Research International, 1, pp. 1–9. doi.org/10.1155/2015/754934.
- Kumar, K., et al (2011) Development of suitable photobioreactors for CO2 sequestration addressing global warming using green algae and cyanobacteria. Bioresource Technology, 102(8), pp. 4945–4953. doi.org/10.1016/j.biortech.2011.01.054.
- Yu, Y., et al. (2013) Development of Synechocystis sp. PCC 6803 as a phototrophic cell factory. Marine Drugs, 11(8), pp. 2894–2916. doi.org/10.3390/md11082894.
- Govindjee, G & Shevela, D (2011) Adventures with cyanobacteria: a personal perspective. Frontiers in Plant Science, 2(28), pp. 1–17. doi.org/10.3389/fpls.2011.00028.
- Tschortner, J., et al. (2019) Biophotovoltaics: Green Power Generation From Sunlight and Water. Frontiers in Microbiology, 10(866), pp. 1–19. doi.org/10.3389/fmicb.2019.00866.
- Van den Heuvel, R. H. H., et al. (2003) The Active Conformation of Glutamate Synthase and its Binding to Ferredoxin. Journal of Molecular Biology, 330(1), pp. 113–128. doi.org/10.1016/S0022-2836(03)00522-9.
- Mayoral, T., et al. (2005) Structural analysis of interactions for complex formation between Ferredoxin-NADP+ reductase and its protein partners. Proteins: Structure, Function, Bioinfomatic, 59(3), pp. 592–602. doi.org/10.1002/prot.20450.
- Kurisu, G., et al. (2003) Structure of the Cytochrome b6f Complex of Oxygenic Photosynthesis: Tuning the Cavity. Science, 302(5647), pp. 1009–1014. doi.org/10.1126/science.1090165.
- Gisriel, C., et al. (2019) Membrane protein megahertz crystallography at the European XFEL. Nature Communications, 10(5021), pp. 1–11. doi.org/10.1038/s41467-019-12955-3.
- Kupitz, C., et al. (2014) Serial time-resolved crystallography of photosystem II using a femtosecond X-ray laser. Nature, 513(7517), pp. 261–265. doi.org/10.1038/nature13453.
- Murphy, B. J., et al. (2019) Rotary substates of mitochondrial ATP synthase reveal the basis of flexible F1-Fo coupling. Science, 364(6446), p. eaaw9128. doi.org/10.1126/science.aaw9128.
- Berman, H., et al. (2003) Announcing the worldwide Protein Data Bank. Nature Structural & Molecular Biology, 10(12), p. 980. doi.org/10.1038/nsb1203-980.
- De Causmaecker, S., et al. (2019) Energetics of the exchangeable quinone, in Photosystem II. Processing of the National Academy of Sciences of the USA, 116(39), pp. 19458–19463. doi.org/10.1073/pnas.1910675116.
- Bottin, H & Lagoutte, B (1992) Ferrodoxin and flavodoxin from the cyanobacterium Synechocystissp PCC 6803. Biochimica et Biophysica Acta (BBA) - Bioenergetics, 1101(1), pp. 48–56. doi.org/10.1016/0167-4838(92)90465-P.
- Caffarri, S., et al. (2014) A comparison between plant photosystem I and photosystem II architecture and functioning. Current Protein & Peptide Science, 15(4), pp. 296–331. doi.org/10.2174/1389203715666140327102218.
- Kothe, T., et al. (2013) Combination of A Photosystem 1-Based Photocathode and a Photosystem 2-Based Photoanode to a Z-Scheme Mimic for Biophotovoltaic Applications. Angewandte Chemie International Edition, 52(52), pp. 14233–14236. doi.org/10.1002/anie.201303671.
- Cassan, N., et al. (2005) Ferredoxin-NADP+ Reductase: Kinetics of Electron Transfer, Transient Intermediates, And Catalytic Activities Studied by Flash-Absorption Spectroscopy with Isolated Photosystem I and Ferredoxin. Journal of Biological Chemistry, 280(28), pp. 25960–25972. doi.org/10.1074/jbc.M503742200.
- Lea-Smith, D. J., et al. (2013) Thylakoid terminal oxidases are essential for the cyanobacterium Synechocystis sp. PCC 6803 to survive rapidly changing light intensities. Plant Physiology, 162(1), pp. 484–495. doi.org/10.1104/pp.112.210260.
- Tiwari, A., et al. (2016) Photodamage of iron-sulphur clusters in photosystem I induces non-photochemical energy dissipation. Nature Plants, 2(4), pp. 1–9. doi.org/10.1038/nplants.2016.35.
- Quintana, N., et al. (2011) Renewable energy from Cyanobacteria: energy production optimization by metabolic pathway engineering. Applied Microbiology and Biotechnology, 91(3), pp. 471–490. doi.org/10.1007/s00253-011-3394-0.
- Choi, O& Sang, B I (2016) Extracellular electron transfer from cathode to microbes: application for biofuel production. Biotechnology Biofuels, 9(1), pp. 1–14. doi.org/10.1186/s13068-016-0426-0.
- Tikkanen, M., et al. (2014) Photosystem II photoinhibition-repair cycle protects Photosystem I from irreversible damage. Biochimica et Biophysica Acta (BBA) - Bioenergetics, 1837(1), pp. 210–215. doi.org/10.1016/j.bbabio.2013.10.001.
- Pospíšil, P (2016) Production of Reactive Oxygen Species by Photosystem II as a Response to Light and Temperature Stress. Frontiers in Plant Science, 7(1), pp. 1–12. doi.org/10.3389/fpls.2016.01950.
- Chan, T., et al. (2012) Quality Control of Photosystem II: Lipid Peroxidation Accelerates Photoinhibition under Excessive Illumination. PLoS One, 7 (12), pp. 1–13. doi.org/10.1371/journal.pone.0052100.
- Ishikawa, Y & Kawai-Yamada, M (2019) Physiological Significance of NAD Kinases in Cyanobacteria. Frontiers in Plant Science, 10(1), pp. 1–13. doi.org/10.3389/fpls.2019.00847.
- Munekage, Y., et al. (2002) PGR5 Is Involved in Cyclic Electron Flow around Photosystem I and Is Essential for Photoprotection in Arabidopsis. Cell, 110(3), pp. 361–371. doi.org/10.1016/S0092-8674(02)00867-X.
- Ungerer, J., et al. (2018) Adjustments to Photosystem Stoichiometry and Electron Transfer Proteins Are Key to the Remarkably Fast Growth of the Cyanobacterium Synechococcus elongatus UTEX 2973. American Society for Microbiology, 9(1), pp. 1–12. doi.org/10.1128/mBio.02327-17.
- McCormick, A. J., et al. (2015) Biophotovoltaics: oxygenic photosynthetic organisms in the world of bioelectrochemical systems. Energy & Environmental Science, 8(4), pp. 1092–1109. doi.org/10.1039/C4EE03875D.
- Bard, A& Faulkner, L (2012) Electrochemical Methods: Fundamentals and Applications, 2nd ed.; John Wiley and Sons, Inc.: New York; p. 823.
- Kracke, F., et al. (2015) Microbial electron transport and energy conservation - the foundation for optimizing bioelectrochemical systems. Frontiers in Microbiology, 6, pp. 1–18. doi.org/10.3389/fmicb.2015.00575.
- Ki, D., et al. (2016) Reduced overpotentials in microbial electrolysis cells through improved design, operation, and electrochemical characterization. Chemical Engineering Journal, 287(1), pp. 181–188. doi.org/10.1016/j.cej.2015.11.022.
- Mershin, A., et al. (2012) Self-assembled photosystem-I biophotovoltaics on nanostructured TiO2and ZnO. Scientific Reports, 2(234), pp. 1–7. doi.org/10.1038/srep00234.
- Wenzel, T., et al. (2018) Porous translucent electrodes enhance current generation from photosynthetic biofilms. Nature Communication, 9(1), pp. 1–9. doi.org/10.1038/s41467-018-03320-x.
- Hasan, K., et al. (2014) Photo-electrochemical communication between cyanobacteria (Leptolyngbia sp.) and osmium redox polymer modified electrodes. Physical Chemistry Chemical Physics, 16(45), pp. 24676–24680. doi.org/10.1039/C4CP04307C.
- Longatte, G., et al. (2018) Investigation of photocurrents resulting from a living unicellular algae suspension with quinones over time. Chemical Science, 9(43), pp. 8271–8281. doi.org/10.1039/C8SC03058H.
- Sawa, M., et al. (2017) Electricity generation from digitally printed cyanobacteria. Nature Communication, 8(1), pp. 1–10. doi.org/10.1038/s41467-017-01084-4.
- Pisciotta, J. M., et al. (2010) Light-dependent electrogenic activity of cyanobacteria. PLoS One, 5(5), pp. 1–10. doi.org/10.1371/journal.pone.0010821.
- Li, Z., et al. (2021) Exogenous electricity flowing through cyanobacterial photosystem I drives CO2valorization with high energy efficiency. Energy Environment Science, 14(10), pp. 5480–5490. doi.org/10.1039/D1EE01526E.
- Kaneko, M., et al. (2017) Cathodic supply of electrons to living microbial cells via cytocompatible redox-active polymers. Electrochemistry Communications, 75(C), pp. 17–20. doi.org/10.1016/j.elecom.2016.12.002.
- Liu, T., et al. (2015) Bioelectricity Generation in a Microbial Fuel Cell with a Self-Sustainable Photocathode. The Scientific World Journal, 2015(1), pp. 1–9. doi.org/10.1155/2015/864568.
- Gorner, H (2004) Photoreactions of p-benzo-, p-naphtho- and p-anthraquinones with ascorbic acid. Photochemical Photobiological Science, 3(10), pp. 933–938. doi.org/10.1039/B410386F.
- Wedege, K., et al. (2016) Organic Redox Species in Aqueous Flow Batteries: Redox Potentials, Chemical Stability and Solubility. Scientific Reports, 6(1), pp. 1–3. doi.org/10.1038/srep39101.
- Flory, J., et al. (2019) Microbial Electro-photosynthesis. US10385304B2. Available at: https://patents.google.com/patent/US10563162B2/en
- Vermaas, W., et al. (1986) Genetically Engineered Mutant of the Cyanobacterium Synechocystis 6803 Lacks thePhotosystem II Chlorophyll-Binding Protein CP-47. Processing of the National Academy of Sciences of the USA, 83(24), pp. 9474–9477. doi.org/10.1073/pnas.83.24.9474.
- Rippka, R., et al. (1979) Generic Assignments, Strain Histories and Properties of Pure Cultures of Cyanobacteria. Microbiology, 111(1), pp. 1–61. doi.org/10.1099/00221287-111-1-1.
- Allen, M M (1968) Simple conditions for growth of unicellular blue-green algae on plates 1, 2. Journal of Phycology, 4(1), pp. 1–4. doi.org/10.1111/j.1529-8817.1968.tb04667.x.
- Lichtenthaler, H K &Wellburn, A R (1983) Determinations of total carotenoids and chlorophylls a and b of leaf extracts in different solvents. Liverpool Meeting, 11(5), pp. 591–592. doi.org/10.1042/bst0110591.
- Zavafer, A., et al. (2015) Photodamage to the oxygen evolving complex of photosystem II by visible light. Scientific Reports, 5(1), p. 16363. doi.org/10.1038/srep16363.
- Murata, N., et al. (2007) Photoinhibition of photosystem II under environmental stress. Biochimica et Biophysica Acta (BBA) - Bioenergetics, 1767(6), pp. 414–421. doi.org/10.1016/j.bbabio.2006.11.019.
- Dobson, Z., et al. (2021) The structure of photosystem I from a high-light-tolerant cyanobacteria. eLife, 10, pp. 1–23. doi.org/10.7554/eLife.67518.
- Nguyen, K., et al. (2017) In vitro kinetics of P700+ reduction of Thermosynechococcus elongatus trimeric Photosystem I complexes by recombinant cytochrome c6 using a Joliot-type LED spectrophotometer. Photosynthesis Research, 131(1), pp. 79–91. doi.org/10.1007/s11120-016-0300-8.
- Latifi, A., et al. (2009) Oxidative stress in cyanobacteria. FEMS Microbiology Reviews, 33(2), pp. 258–278. doi.org/10.1111/j.1574-6976.2008.00134.x.
- Ledford, H K&Niyogi, K K (2005) Singlet oxygen and photo-oxidative stress management in plants and algae. Plant, Cell & Environment, 28(8), pp. 1037–1045. doi.org/10.1111/j.1365-3040.2005.01374.x.
- White, C. C., et al. (1978) Duroquinol as an electron donor for chloroplast electron transfer reactions. Biochimica et Biophysica Acta (BBA) - Bioenergetics, 502(1), pp. 127–137. doi.org/10.1016/0005-2728(78)90137-8.
- Nanba, M & Katoh, S (1986) The site and mechanism of duroquinol oxidation by the cytochrome b6-f complex in Synechococcus sp. Biochimica et Biophysica Acta (BBA) - Bioenergetics, 851(3), pp. 484–490. doi.org/10.1016/0005-2728(86)90085-X.
- Schuurmans, R. M., et al. (2014) The redox potential of the plastoquinone pool of the cyanobacterium Synechocystis species strain PCC 6803 is under strict homeostatic control. Plant Physiology, 165(1), pp. 463–475. doi.org/10.1104/pp.114.237313.
- Peters, J. W., et al. (2016) Electron bifurcation. Current Opinion in Chemical Biology, 31(1), pp. 146–152. doi.org/10.1016/j.cbpa.2016.03.007.
- Cordara, A., et al. (2018) Analysis of the light intensity dependence of the growth of Synechocystis and of the light distribution in a photobioreactor energized by 635 nm light. PeerJ- Biochemistry, Biophysics and Molecular Biology, 6, pp. 1–28. doi.org/10.7717/peerj.5256.