Gas-phase spectroscopy has been a highly active area of development for many years. Many of the earliest developments in spectroscopy in the 18th century involved measurements on gaseous systems, with some of the most famous work being Joseph Ritter von Fraunhofer’s discovery of the dark absorption lines of the Sun and his development of the use of diffraction gratings for spectroscopy in 1821.1
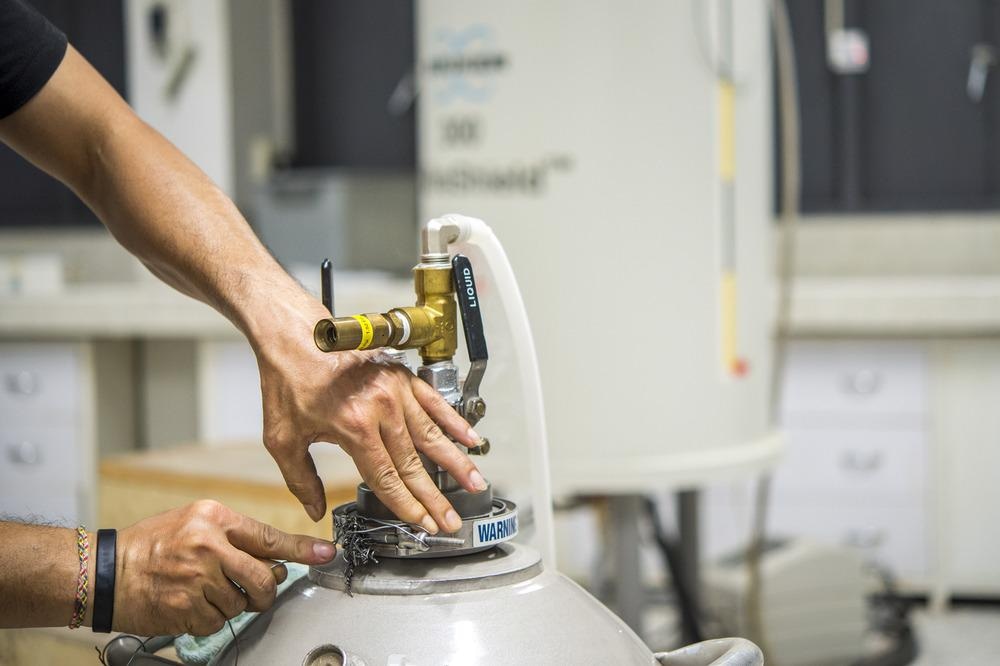
Image Credit: Rabbitmindphoto/Shutterstock.com
Much of the early work on atomic spectroscopy also continued on gaseous systems. Gustav Robert Kirchoff and Robert Wilhelm Eberhard von Bunsen were the first to systematically study how the emission lines varied in wavelength depending on the element of interest by burning various chemicals in a flame. This discovery has formed the basis of atomic emission spectroscopy (AES), a technique that is still widely used today for the elemental analysis of samples.
The development of optics technologies and light sources such as lasers has meant a wealth of new spectroscopic techniques and more complex detection schemes are now available. These developments have led to new possibilities in gas-phase spectroscopy for trace gas detection, real-time sensing, and very high-resolution measurements that have revealed new insights into how chemical reactivity between different types of species work.2
High-resolution gas-phase measurements have also proved extremely important for the validation of many theoretical techniques and developing our understanding of molecular chemistry at the atomic level.3
Number Densities
Gases are often a very challenging state of matter to deal with for spectroscopic techniques because, relative to solids and liquids, gases have very low number densities – the number of atomics or molecules per unit volume.
As the signal observed in a spectroscopic measurement relies on an interaction between the incident radiation and species of interest, the signal strength is typically proportional to the number of molecules interacted with. This is what makes single-molecule spectroscopies so challenging to perform.4
While using larger beam sizes to compensate for the number density issue may seem desirable, it is often not practical. Expanding the output beam of a light source over a larger area usually involves a trade-off between the power density and volume of illumination. Many sources are simply not powerful enough to be used with larger spot sizes, particularly for non-linear spectroscopy applications that require high peak powers.
However, from a scientific perspective, the low number density of gaseous samples does come with some advantages. When the number density is sufficiently low, it can be assumed that the molecules in the gas of interest have little to no interactions with each other and so the substance of interest can be studied without a perturbing environment to affect its properties.
The lack of interaction with an environment also leads to inherently narrower linewidths. Molecular species in interacting environments tend to have a significant contribution of inhomogeneous broadening to their linewidths, resulting in much broader peaks than isolated systems. Under the right experimental conditions, it is possible to eliminate many of the homogenous broadening contributions to the lineshapes of gas-phase molecules and perform incredibly high precision measurements that can recover the ‘natural’ linewidth for a given transition, which corresponds to its lifetime.
Absorption and Charged Particle Detection
One experimental approach to circumvent the issue of low number densities in gases has been to use incredibly long pathlengths by passing the incident beam many times through the sample, such as in cavity ring-down experiments.5 This is one way of achieving trace gas detection and has been widely used to probe difficult-to-generate species such as radicals for atmospheric chemistry research.
Like many gas-phase spectroscopic techniques, cavity ring-down measurements rely on the absorption of light by the molecule. Whereas UV-visible absorption spectroscopy can be difficult to use as an identification method for molecules in solution due to absorption bands generally being very broad and featureless, in the gas phase, many molecules have sharp features in the electronic absorption spectra that can be used to ‘fingerprint’ the molecular species of interest.
Emission spectroscopy, where photons are emitted from a photoexcited sample, is a very popular technique for condensed phase samples. However, in the gas phase, particularly for experiments being performed under high-vacuum conditions, it can be challenging to collect sufficient photons to achieve a good signal to noise in the resulting spectrum.
Instead, many gas-phase experiments used charged particle detection methods to detect the electrons or ions emitted from the molecule after it interacts with light. Photoelectron spectroscopy methods have been widely used to study the electronic structure of gaseous molecules as, if the experiment is performed under suitably high vacuum conditions, it is reasonable to assume the detected electrons have not undergone any collisions with other species and so their kinetic energy reflects the binding energy of the molecular orbital from which they originated.
Benefitting from higher power sources and more sensitive detectors, spectroscopic measurements of gases will continue to be a very powerful tool for fundamental research6 but also for highly practical use, such as air quality monitoring and gas leak detection.
References and Further Reading
- Brand, John C. D. (1995). Lines of Light: The Sources of Dispersive Spectroscopy, 1800–1930. Gordon and Breach Publishers. pp. 37–42. ISBN 978-2884491624.
- Meyer, J., & Wester, R. (2017). Ion – Molecule Reaction Dynamics. Annual Reviews of Physical Chemistry, 68, 333–353. https://doi.org/10.1146/annurev-physchem-052516-044918
- Puzzarini, C., & Barone, V. (2018). Diving for Accurate Structures in the Ocean of Molecular Systems with the Help of Spectroscopy and Quantum Chemistry. Accounts of Chemical Research, 51, 548–556. https://doi.org/10.1021/acs.accounts.7b00603
- Moerner, W. E., Schechtman, Y., & Wang, Q. (2015). Single-Molecule Spectroscopy and Imaging Over the Decades. Faraday Discussions, 184, 9–36. https://pubs.rsc.org/en/content/articlelanding/2015/FD/C5FD00149H
- Moerner, W. E., Schechtman, Y., & Wang, Q. (2015). Single-Molecule Spectroscopy and Imaging Over the Decades. Faraday Discussions, 184, 9–36. https://pubs.rsc.org/en/content/articlelanding/2015/FD/C5FD00149H
- Zettergren, H., Domaracka, A., Schlath, T., Labuda, M., Tosic, S., Maclot, S., Johnsson, P., Steber, A., Castrovilli, M. C., Avaldi, L., Bari, S., & Milosavljevi, A. R. (2021). Roadmap on dynamics of molecules and clusters in the gas phase. The European Physical Journal DEuropean Physics Journal D, 75, 152. https://doi.org/10.1140/epjd/s10053-021-00155-y
Disclaimer: The views expressed here are those of the author expressed in their private capacity and do not necessarily represent the views of AZoM.com Limited T/A AZoNetwork the owner and operator of this website. This disclaimer forms part of the Terms and conditions of use of this website.