The upcoming Extremely Large Telescopes (ELTs) are likely to possess enough collecting area to identify potential biosignature gases such as molecular oxygen (O2) present in terrestrial planet atmospheres orbiting nearby stars.
Transmission spectroscopy is considered one of the most effective detection methods. To enhance our ability to detect O2 using this approach, spectral resolutions of at least R ≥ 300,000 are necessary to fully distinguish the absorption lines in an Earth-like exoplanet atmosphere and separate the signal from telluric lines.
Current high-resolution spectrographs typically achieve spectral resolutions around R ∼ 100,000. However, increasing the resolution in seeing-limited observations and instruments demands significantly larger optical components, making these instruments costly and challenging to manufacture and assemble.
In this article, a different method for achieving high-resolution spectroscopy is demonstrated. An ultra-high spectral resolution booster has been incorporated into a high-resolution spectrograph, utilizing a chained Fabry–Perot array to generate a hyperfine spectral profile.
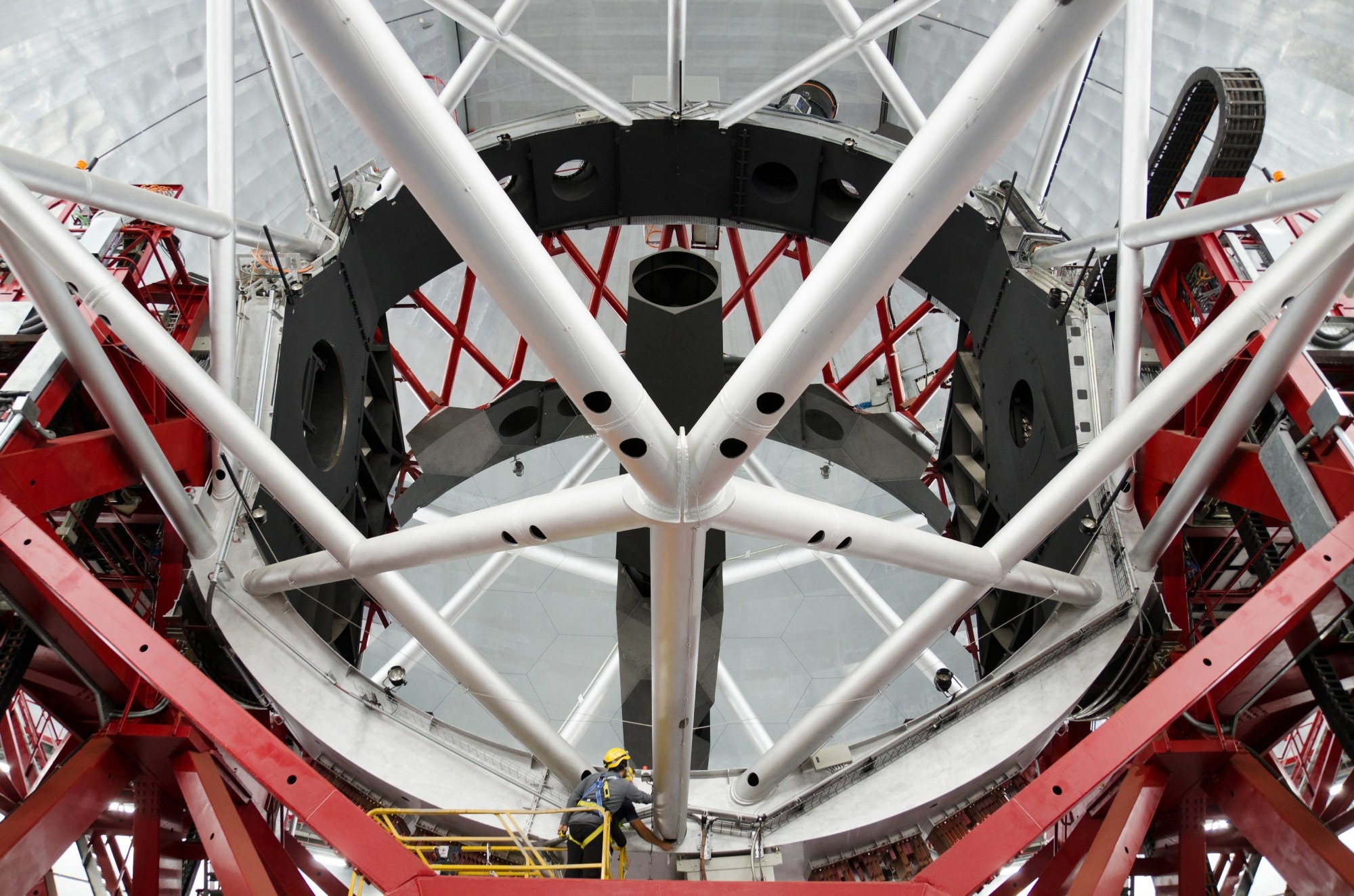
Image Credits: David Herraez Calzada/Shutterstock.com
On-sky telluric observations with a laboratory demonstrator are also presented. Depending on the setup, this two-arm prototype achieves resolutions ranging from R=250,000 to R=350,000. Following a thorough modeling of the prototype's behavior, a design proposal for a Fabry–Perot Interferometer (FPI) with an eight-arm array configuration is suggested for Extremely Large Telescopes (ELTs), aiming to surpass a resolution of R=300,000.
The innovative FPI resolution booster can be integrated at the front end of an existing R=100,000 spectrograph to replace the spectral profile with a higher resolution, specifically for studies on exoplanet atmospheres.
In the last approximately 25 years, a few confirmed exoplanets, commonly called terrestrial exoplanets, have been identified with a rocky composition.
Missions like Kepler and TESS have revealed that these planets are abundant in the solar neighborhood.4,13,41 A logical progression in investigating terrestrial exoplanets involves understanding their atmospheres, a topic that remains largely uncertain.60
Places in the habitable zone are particularly intriguing, where their orbits permit liquid water on the surface. Examining the atmospheres of these planets provides a broader context for Earth and helps us comprehend the prevalence of conditions conducive to life's evolution on our home planet.
The potential identification of biomarkers in such studies presents an exciting opportunity for the scientific community.
The most common and extensive studies of exoplanet atmospheres commonly occur through transit spectroscopy, wherein the chemical composition of exoplanet atmospheres is examined using spectra collected during transits.10,48 As a planet moves across the observer's line of sight to its host star, the atmospheric molecules leave distinctive marks on the spectrum through absorption lines.
The exoplanet atmosphere's geometric cross section is significantly smaller compared to the star's surface area (10−4 - 10−6), underscoring the importance of high signal-to-noise ratio (S/N) observations in this method.
Recent research has identified various species in the atmospheres of gas giants using high-resolution transmission spectroscopy. For instance, CO,8,11,43,51 H2O,2,7 and TiO23 have been detected through techniques such as cross-correlation51 and Doppler tomography.59
These studies indicate that high-resolution spectra (R ≥ 100,000) are advantageous for identifying molecules in an exoplanet's atmosphere. At a fixed S/N per resolution element, a higher resolving power instrument can investigate fainter and narrower spectral lines.3
An intriguing molecule that has not yet been observed in an exoplanet atmosphere is molecular oxygen (O2). O2 holds potential significance as an indicator of biological activity on exoplanets, using Earth as a reference.46
While it is crucial to interpret O2 within its environmental context, this molecule remains a significant biosignature, and advancing the instrumental capability for its detection is a vital aspect of the quest for life beyond the Solar System.22,29,35
On Earth, oxygenic photosynthesis is the source of O2.34 Tracing back to the origin of life, the first single-celled organisms, cyanobacteria, initiated the conversion of carbon dioxide (CO2) into O2 through photosynthesis.24
During the Archean Eon (2.5 − 4 billion years ago), Earth's atmosphere became enriched with O2, likely contributing to the emergence of diverse flora and fauna. The rapid bonding of atomic oxygen with other molecules necessitates regular replenishment to maintain the high concentration of O2 observed in the atmosphere.
Given that O2 is a plentiful gas uniformly distributed in the atmosphere, it is accessible for remote detection using methods such as transit spectroscopy.35
Although the presence of O2 in exoplanet atmospheres could stem from non-biological processes, identifying O2 can serve as a robust indicator of biological activity when considered in the broader stellar and planetary context.12,17,21,30,35,61
Detecting O2 in Earth-like exoplanet atmospheres through space-based missions within the next decade appears unlikely.
The limited instrumental sensitivity and resolution of JWST at shorter wavelengths are likely to hinder detection in the primary O2 absorption bands, such as the B-band (650 nm), A-band (765 nm), and the near-infrared (NIR) (1270 nm).62
A recently proposed alternative approach for O2 detection at 6.4 µm by Fauchez et al. presents an intriguing option, but the likelihood of detection through Earth-based observations seems more feasible.15
As the next-generation Giant Segmented Mirror Telescopes (GSMTs) start coming online, the expectation is that their extensive collecting areas will enable the high signal-to-noise ratio (S/N) observations needed to identify molecular oxygen in the atmospheres of terrestrial exoplanets.42,52
Various instruments with high dispersion capabilities, including G-CLEF,55 GMTNIRS,25 METIS,6 and HIRES/ANDES,32 could contribute to examining exoplanet atmospheres and potentially detecting O2.
These instruments achieve a maximum resolution of R=150,000. According to López-Morales et al., ultrahigh-resolution in the range of R=300,000-400,000 is optimal for O2 detection in the atmospheres of terrestrial exoplanets.28
This determination is influenced by line-of-sight effects and the expected narrow line profile resulting from refraction effects by lower layers in an exoplanet atmosphere. Moreover, at R ≥ 300,000, it becomes feasible to separate the Earth's telluric spectrum from the exoplanet's atmospheric spectrum by leveraging the relative velocity of the latter.
Seeing-limited high-resolution Echelle spectrographs face limitations in achieving resolutions significantly higher than ∼100,000 for apertures ranging from 6.5 to 10 meters due to the conservation of etendue.
A large collecting area implies a larger collimated beam, dispersion grating, and instrument as a whole. The challenge is magnified when considering Giant Segmented Mirror Telescopes (GSMTs), and the dependence of the resolution on the telescope diameter for such instruments (R ∝ 1/D).47
As a result, a novel instrument approach is imperative to enhance spectral resolution, and significant efforts are underway for this purpose.
The precision radial velocity (PRV) community has devised methods such as pupil or image slicers to elevate spectral resolution, as seen in instruments like G-CLEF,55 CARMENES,40 PEPSI,54 FIDEOS,55 and TARdYS.45
This solution encounters limitations due to the available detector area on the spectrograph focal plane.
Another alternative involves the use of externally dispersed spectrographs to achieve exceptionally high resolutions, exemplified by Doppler exoplanet surveys conducted using the Dispersed Fixed Delay Interferometer (DFDI) and the TripleSpec Exoplanet Discovery Instrument (TEDI).14,19,20
DFDI optimizes optical delay in the interferometer and mitigates photon noise by measuring multiple fringes across a broad spectrum. This interferometer is then coupled with a low- to medium-resolution post-disperser.18
On the other hand, TEDI employs externally dispersed interferometry (EDI) in conjunction with a conventional high-resolution R=20,000 spectrograph.
The prototype showcased a sixfold increase in resolving power, but this gain comes at the expense of exposure time, as it is possible to sample only a limited subspace of the frequency range covered by the signal in Fourier space.14
Considering these outcomes and challenges, Ben-Ami et al. proposed a resolution booster for high-resolution spectrographs. The Fabry–Perot Instrument for Oxygen Searches (FIOS) concept involves a Fabry–Perot interferometer (FPI) array that generates an ultra-high-resolution spectral transmission profile.1
When integrated with an existing high-resolution spectrograph, FIOS can enhance the spectral resolving power of the instrument to the level optimal for O2 detection. A laboratory demonstrator of FIOS featuring two etalons or dualons was presented by Rukdee et al.44
This article describes the initial on-sky observations of the Sun using the FIOS laboratory demonstrator while connected to a solar telescope.
Conclusion
This study presents an experimental demonstration of the two-arm prototype (equivalent to one-fourth of a full chain) of FIOS, showcasing its ability to capture a telluric spectrum at a high resolution of R=250,000 and to characterize narrow O2 spectral features under realistic conditions.
The addition of an extra arm proved beneficial in enhancing the sampling frequency and overall resolution towards the upper limit of R from the laser scan measurement, indicating positive prospects for the future advancement of FIOS.
The interconnected ultra-high-resolution dualon array undergoes cross-dispersion by a high-resolution spectrograph with spectral resolution.
By integrating across the transmission peaks resolved by the external spectrograph, the study successfully emulates the behavior of a lower-resolution spectrograph, matching the resolution similar to the dualons' Free Spectral Range (FSR).
This emulation is demonstrated to be sufficient to unlock an ultra-high resolution for the instrument, making FIOS a plug-in resolution booster for a narrow band. The minimum requirement for the external spectrograph's compatibility is R = 100,000, equivalent to 1 FSR of the Fabry–Perot Interferometer (FPI).
The selection of multi-mode fiber was made to ensure a reasonable throughput and aperture ratio among the optical components in the system. In natural seeing conditions, the external spectrograph on Extremely Large Telescopes (ELTs) will utilize multi-mode large core size fiber.
This choice makes this study's demonstration realistic for the envisioned future implementation of FIOS on ELTs. Beyond O2, the concept can extend to the study of other spectral bands and lines, such as methane (CH4), where the lines exhibit higher density than the oxygen band.
This approach holds promise for investigating future exoplanet atmospheres by integrating FIOS into upcoming ELTs like G-CLEF,54 ANDES,32 and MODHIS,33 with minimal alterations from the original concepts of these instruments.
Download White Paper to Learn More
References and Further Reading
- Ben-Ami, S., López-Morales, M., Garcia-Mejia, J., Gonzalez Abad, G., & Szentgyorgyi, A. 2018, ApJ, 861, 79
- Birkby, J. L., de Kok, R. J., Brogi, M., Schwarz, H., & Snellen, I. A. G. 2017, AJ, 153, 138
- Black, J. 2005, in High Resolution Infrared Spectroscopy in Astronomy, 3–14
- Borucki, W. J. 2010, in APS Meeting Abstracts, Vol. 2010, APS April Meeting Abstracts, V1.002
- Bourdarot, G., Le Coarer, E., Alecian, E., Rabou, P., & Bonfils, X. 2017, in Society of Photo-Optical Instrumentation Engineers (SPIE) Conference Series, Vol. 10562, Society of Photo-Optical Instrumentation Engineers (SPIE) Conference Series, 105622D
- Brandl, B. R., Feldt, M., Glasse, A., et al. 2014, in Society of Photo-Optical Instrumentation Engineers (SPIE) Conference Series, Vol. 9147, Groundbased and Airborne Instrumentation for Astronomy V, ed. S. K. Ramsay, I. S. McLean, & H. Takami, 914721
- Brogi, M., de Kok, R. J., Birkby, J. L., Schwarz, H., & Snellen, I. A. G. 2014, Astronomy & Astrophysics, 565, A124
- Brogi, M., Snellen, I. A. G., de Kok, R. J., et al. 2012, Nature, 486, 502
- Cersullo, F., Wildi, F., Chazelas, B., & Pepe, F. 2017, A new infrared FabryPérot-based radial-velocity-reference module for the SPIRou radial-velocity spectrograph
- Charbonneau, D., Brown, T. M., Noyes, R. W., & Gilliland, R. L. 2002, The Astrophysical Journal, 568, 377
- de Kok, R., Brogi, M., Snellen, I., et al. 2013, Astronomy & Astrophysics, 554, A82
- Domagal-Goldman, S. D., Segura, A., Claire, M. W., Robinson, T. D., & Meadows, V. S. 2014, ApJ, 792, 90
- Dressing, C. D. & Charbonneau, D. 2015, ApJ, 807, 45
- Edelstein, J., Muterspaugh, M. W., Erskine, D. J., et al. 2007, in Techniques and Instrumentation for Detection of Exoplanets III, ed. D. R. Coulter, Vol. 6693, International Society for Optics and Photonics (SPIE), 276 – 281
- Fauchez, T. J., Villanueva, G. L., Schwieterman, E. W., et al. 2020, Nature Astronomy, 4, 372–376
- Foreman-Mackey, D., Hogg, D. W., Lang, D., & Goodman, J. 2013, PASP, 125, 306
- Gao, P., Hu, R., Robinson, T. D., Li, C., & Yung, Y. L. 2015, ApJ, 806, 249
- Ge, J. 2002, ApJ, 571, L165
- Ge, J., Erskine, D. J., & Rushford, M. 2002, PASP, 114, 1016
- Ge, J., van Eyken, J., Mahadevan, S., et al. 2006, The Astrophysical Journal, 648, 683
- Harman, C. E., Schwieterman, E. W., Schottelkotte, J. C., & Kasting, J. F. 2015, ApJ, 812, 137
- Hitchcock, D. R. & Lovelock, J. E. 1967, Icarus, 7, 149
- Hoeijmakers, H. J., de Kok, R. J., Snellen, I. A. G., et al. 2015, Astronomy & Astrophysics, 575, A20
- Holland, H. D. 2006, Philosophical Transactions of the Royal Society B: Biological Sciences, 361, 903–915
- Jaffe, D. T., Barnes, S., Brooks, C., et al. 2016, in Society of Photo-Optical Instrumentation Engineers (SPIE) Conference Series, Vol. 9908, Ground-based and Airborne Instrumentation for Astronomy VI, ed. C. J. Evans, L. Simard, & H. Takami, 990821
- Jones, A., Noll, S., Kausch, W., Szyszka, C., & Kimeswenger, S. 2013, A&A, 560, A91
- Jurgenson, C., Fischer, D., McCracken, T., et al. 2016, in Society of PhotoOptical Instrumentation Engineers (SPIE) Conference Series, Vol. 9908, Ground-based and Airborne Instrumentation for Astronomy VI, ed. C. J. Evans, L. Simard, & H. Takami, 99086T
- López-Morales, M., Ben-Ami, S., Gonzalez-Abad, G., et al. 2019, AJ, 158, 24
- Lovelock, J. E. 1965, Nature, 207, 568
- Luger, R. & Barnes, R. 2015, Astrobiology, 15, 119
- Marconi, A., Abreu, M., Adibekyan, V., et al. 2022, in Society of Photo-Optical Instrumentation Engineers (SPIE) Conference Series, Vol. 12184, Groundbased and Airborne Instrumentation for Astronomy IX, ed. C. J. Evans, J. J. Bryant, & K. Motohara, 1218424
- Marconi, A., Allende Prieto, C., Amado, P. J., et al. 2018, in Society of PhotoOptical Instrumentation Engineers (SPIE) Conference Series, Vol. 10702, Ground-based and Airborne Instrumentation for Astronomy VII, ed. C. J. Evans, L. Simard, & H. Takami, 107021Y
- Mawet, D., Fitzgerald, M., Konopacky, Q., et al. 2019, in Bulletin of the American Astronomical Society, Vol. 51, 134
- Meadows, V. S. 2017, Astrobiology, 17, 1022
- Meadows, V. S., Reinhard, C. T., Arney, G. N., et al. 2018, Astrobiology, 18, 630
- Mello, A. J. T. S., Bouchez, A. H., Szentgyorgyi, A., van Dam, M. A., & Lupinari, H. 2018, MNRAS, 481, 3804
- Moehler, S., Modigliani, A., Freudling, W., et al. 2014, A&A, 568, A9
- Noll, S., Kausch, W., Barden, M., et al. 2012, A&A, 543, A92
- Pepe, F., Molaro, P., Cristiani, S., et al. 2014, Astronomische Nachrichten, 335, 8
- Quirrenbach, A., Amado, P. J., Caballero, J. A., et al. 2014, in Society of PhotoOptical Instrumentation Engineers (SPIE) Conference Series, Vol. 9147, Ground-based and Airborne Instrumentation for Astronomy V, ed. S. K. Ramsay, I. S. McLean, & H. Takami, 91471F
- Ricker, G. R., Winn, J. N., Vanderspek, R., et al. 2014, in Society of PhotoOptical Instrumentation Engineers (SPIE) Conference Series, Vol. 9143, Space Telescopes and Instrumentation 2014: Optical, Infrared, and Millimeter Wave, ed. J. Oschmann, Jacobus M., M. Clampin, G. G. Fazio, & H. A. MacEwen, 914320
- Rodler, F. & López-Morales, M. 2014, The Astrophysical Journal, 781, 54
- Rodler, F., Lopez-Morales, M., & Ribas, I. 2012, ApJ, 753, L25
- Rukdee, S., Ben-Ami, S., Szentgyorgyi, A., et al. 2020, in Ground-based and Airborne Instrumentation for Astronomy VIII, ed. C. J. Evans, J. J. Bryant, & K. Motohara, Vol. 11447, International Society for Optics and Photonics (SPIE), 337 – 350
- Rukdee, S., Vanzi, L., Schwab, C., et al. 2019, Experimental Astronomy, 48, 145
- Sagan, C., Thompson, W. R., Carlson, R., Gurnett, D., & Hord, C. 1993, Nature, 365, 715
- Schroeder, D. J. 2000, Astronomical Optics
- Seager, S. & Sasselov, D. D. 2000, ApJ, 537, 916
- Seifahrt, A., Bean, J. L., Kasper, D., et al. 2022, in Society of Photo-Optical Instrumentation Engineers (SPIE) Conference Series, Vol. 12184, Groundbased and Airborne Instrumentation for Astronomy IX, ed. C. J. Evans, J. J. Bryant, & K. Motohara, 121841G
- Shirasaki, M. 1996, Optics Letters, 21, 366
- Snellen, I. A. G., de Kok, R. J., de Mooij, E. J. W., & Albrecht, S. 2010, Nature, 465, 1049
- Snellen, I. A. G., de Kok, R. J., le Poole, R., Brogi, M., & Birkby, J. 2013, ApJ, 764, 182
- Sofer Rimalt, Y., Ben Ami, S., Mazeh, T., et al. 2022, in Society of Photo-Optical Instrumentation Engineers (SPIE) Conference Series, Vol. 12184, Groundbased and Airborne Instrumentation for Astronomy IX, ed. C. J. Evans, J. J. Bryant, & K. Motohara, 1218459
- Strassmeier, K. G., Ilyin, I., Järvinen, A., et al. 2015, Astronomische Nachrichten, 336, 324
- Szentgyorgyi, A., Baldwin, D., Barnes, S., et al. 2016, in Proc. SPIE, Vol. 9908, Ground-based and Airborne Instrumentation for Astronomy VI, 990822
- Vanzi, L., Zapata, A., Flores, M., et al. 2018, MNRAS, 477, 5041
- Vaughan, J. 1989, The Fabry-Perot Interferometer: History, Theory, Practice and Applications
- Wallace, L., Hinkle, K. H., Livingston, W. C., & Davis, S. P. 2011, ApJS, 195, 6
- Watson, C. A., de Mooij, E. J. W., Steeghs, D., et al. 2019, Monthly Notices of the Royal Astronomical Society, 490, 1991–2006
- Wordsworth, R. & Kreidberg, L. 2022, Annual Review of Astronomy and Astrophysics, 60, null
- Wordsworth, R. & Pierrehumbert, R. 2014, ApJ, 785, L20
- Wunderlich, F., Godolt, M., Grenfell, J. L., et al. 2019, A&A, 624, A49
- Zhu, X., Lin, D., Hao, Z., Wang, L., & He, J. 2020, The Astronomical Journal, 160, 135
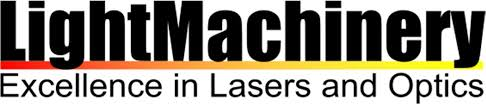
This information has been sourced, reviewed and adapted from materials provided by LightMachinery Inc.
For more information on this source, please visit LightMachinery Inc.